The content of this book is licensed under a Creative Commons Attribution-NonCommercial-NoDerivs 4.0 Unported license. To view the terms and conditions of this license, visit https://creativecommons.org/licenses/by-nc-nd/4.0/
NCBI Bookshelf. A service of the National Library of Medicine, National Institutes of Health.
Varki A, Cummings RD, Esko JD, et al., editors. Essentials of Glycobiology [Internet]. 4th edition. Cold Spring Harbor (NY): Cold Spring Harbor Laboratory Press; 2022. doi: 10.1101/glycobiology.4e.57
Several classes of successful commercial products are based on isolated or synthetic glycans or agents that alter their expression and recognition. This chapter summarizes the use of glycans as vaccines and therapeutics. Applications of glycan mimics as drugs are also discussed.
GLYCANS AS COMPONENTS OF SMALL-MOLECULE DRUGS
Many well-known small-molecule drugs, such as antibiotics and anticancer therapeutic agents, are natural products that contain glycans as part of their core structure and/or as a sugar side chain (i.e., a glycoside). Some modern examples of natural products that bear glycan side chains are shown in Figure 57.1. The well-established area of natural product chemistry will not be reviewed in detail here, though many natural products are glycosylated such as the well-known digitalis. Modified glycans gave rise to synthetic drugs such as small-molecule inhibitors of influenza virus neuraminidase (see also Chapter 55). Recent advances in the functional understanding of carbohydrate–protein interactions have enabled the development of glycomimetics, a new class of small-molecule drugs that are briefly described.
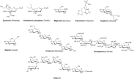
FIGURE 57.1.
Examples of natural products that contain glycan components. Streptomycin and erythromycin A are antibiotics, doxorubicin is chemotherapeutic drug, and digoxin is used to treat cardiovascular disease.
Rational Drug Design—Small-Molecule Inhibitors of Influenza Virus Neuraminidase
Influenza virus has two major surface proteins, hemagglutinin and neuraminidase (see Chapter 34). The hemagglutinin initiates infection by binding to cell-surface sialic acids. The neuraminidase assists virus release by cleaving sialic acids to prevent unwanted retention of newly synthesized virus on the cell surface. Neuraminidase may also function during the invasion phase by removing sialic acids on soluble mucins that would otherwise inhibit cell-surface binding. Because neuraminidase is essential to the viral life cycle, based on the crystal structure of the enzyme a rational drug design program yielded zanamivir (Relenza). The addition of a bulky guanidino side chain at C-4 of a previously known neuraminidase inhibitor, 2-deoxy-2,3-dehydro-N-acetyl-neuraminic acid, markedly increased the affinity for influenza neuraminidase, without affecting host-cell neuraminidases (Figure 57.2; see also Chapter 55). Relenza blocks the influenza virus life cycle by interrupting the spread of the virus during the early phase of an infection and subsequently preventing infection. Because of poor oral availability, Relenza has to be inhaled to work at the mucosal sites of infection in the upper airway. Because of the ease of use the orally available drug oseltamivir (Tamiflu) achieves the same effects and has taken over most of the market.
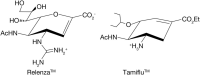
FIGURE 57.2.
The synthetic influenza neuraminidase inhibitors Relenza and Tamiflu.
The fear of avian influenza virus (“bird flu”) spreading into human populations has prompted stockpiling of Tamiflu. Fortunately, widespread use of the drug has not become necessary to date. The development of Tamiflu is a textbook example for rational glycan-based drug design resulting in a powerful drug against a devastating disease.
THERAPEUTIC GLYCOPROTEINS
Most biotherapeutic products are glycoproteins, including erythropoietin, various other cytokines, antibodies, glycosyltransferases, and glycosidases. This class of molecules sells in the tens of billions of U.S. dollars annually worldwide. Therapeutic glycoproteins or glycan-processing/recognizing proteins are typically produced recombinantly in cell culture systems or, less commonly, in the milk of transgenic animals. Control of glycosylation is of major importance during the development of these drugs, because their glycan chains have marked effects on stability, activity, antigenicity, and pharmacodynamics in intact organisms. Frequently, glycosylation must be optimized to ensure prolonged circulatory half-life in the blood. Manipulation of glycans to promote targeting to specific tissues and cell types has also been a useful element of drug design.
Optimizing Glycans of Therapeutic Glycoproteins for Prolonged Serum Half-Life
Erythropoietin (EPO) is the most successful biotechnology product to date. It is a circulating cytokine that binds to the erythropoietin receptor, inducing proliferation and differentiation of erythroid progenitors in the bone marrow. EPO was developed to treat anemias caused by bone marrow suppression after chemotherapy or lack of erythropoietin (e.g., renal failure). Natural and recombinant forms of erythropoietin carry three sialylated complex N-glycans and one sialylated O-glycan. Although in vitro the activity of deglycosylated erythropoietin is comparable to that of the fully glycosylated molecule, its activity in vivo is reduced by ∼90%, because poorly glycosylated EPO is rapidly cleared by filtration in the kidney. Undersialylated EPO is also rapidly cleared by galactose receptors in hepatocytes and macrophages (see Chapter 31). Fully sialylated chains and increased tetra-antennary branching reduce these problems and increase EPO activity in vivo nearly tenfold. Addition of an N-glycosylation site also increases half-life and activity in vivo. Covalently linking polyethylene glycol to the protein also reduces clearance by the kidney.
Erythropoietin is unusual because it is small enough to be cleared by the kidney if it is underglycosylated. For most glycoprotein therapeutics, a more important consideration is minimizing clearance by galactose-binding hepatic receptors by ensuring full sialylation of glycans. Glycans greatly influence the efficacy of these drugs; therefore, controlling glycosylation during production is crucial considering regulatory requirements for batch-to-batch product consistency. Changes in culture pH, the availability of precursors and nutrients, and the presence or absence of various growth factors and hormones can each affect the extent of glycosylation, the degree of branching, and the completeness of sialylation. Sialidases and other glycosidases that are either secreted or released by dead cells can also degrade the previously intact product in the culture medium. These issues were hotly debated with the advent of “biosimilars” or generic versions of glycoproteins. The need to prove composition has fueled efforts devoted to glycan analysis and sequencing.
Impact of Glycosylation on Licensing and Patentability of Biotherapeutic Agents
Patenting new therapeutics is typically based on the composition of matter in the claimed molecule. Small molecules of defined structure and nonglycosylated proteins are easily captured in this manner. However, glycoproteins, especially those with multiple glycosylation sites, render it virtually impossible to obtain preparations that contain only a single glycoform. For examples of glycosylated therapeutics, please see Table 57.1. Thus, most biotherapeutic glycoproteins consist of a mixture of glycoforms. Licensing bodies allow for a certain range of variation in glycoforms and complexity of the mixture. However, the manufacturer and the agency must agree on the extent such variation is acceptable for a given drug formulation. Biopharmaceutical companies therefore spend considerable effort in assuring that their products fall within these defined ranges, once these are approved by licensing bodies. The inherent difficulty in reproducing complex glycoform mixtures also complicates efforts to make generic forms of recombinant glycoprotein drugs. Given the complexities of producing glycotherapeutic agents in mammalian cells, licensing agencies use consistency in glycoform composition as an indirect measure of the quality of process control in production. Glycosylation differences can have implications for the patentability of agents in which the polypeptide remains constant. Marked glycosylation differences have been used to define agents as being unique. However, it is usually necessary to show that the differences in glycosylation being claimed have a correspondingly significant effect in changing the functionality of the drug in question. The associated pharmaceutical licensing and legal issues are rapidly evolving to keep pace with scientific advances in this area.
TABLE 57.1.
Examples of glycan-based drugs, their target diseases, and modes of action
GLYCOSYLATION ENGINEERING
There are limits as to how much of a biotherapeutic glycoprotein an animal cell line can produce. Production becomes an issue in cases where very large amounts of a particular glycoprotein are needed. Glycoprotein production in plants or yeast is attractive but makes it necessary to eliminate risks arising from the nonhuman glycans of plant and fungal cells that could cause excessively rapid clearance and/or antigenic reactions. Many plant and yeast glycans are immunogenic and elicit glycan-specific IgE and IgG antibodies in humans when delivered parenterally. A variety of mammalian genes have been introduced into yeast and/or genes that are producing nonhuman glycosylation have been eliminated. Extensively engineered yeast strains are capable of producing biantennary N-linked glycans with the human sialic acid N-acetylneuraminic acid (Neu5Ac) but the productivity of such yeast strains is often low. Efforts to engineer yeast to make human-like O-glycans are underway.
Plants and algae as well as insect cell lines have also been used to engineer recombinant glycoproteins, but, as in yeast, the glycans produced by plants differ from those found in vertebrates. The antigenic differences that arise in recombinant glycoproteins produced in plants become less problematic if used for topical or oral administration, because humans are normally exposed to plant glycans in the diet. The cost of production is much lower than in animal cell culture systems and animal sera are not needed. As in yeast, “humanizing plants” with respect to glycosylation may allow the production of nonimmunogenic glycoproteins. Chemical methods for synthesizing entire glycoproteins from scratch have been developed and single glycoforms of EPO have been prepared by total synthesis. Given the complexity of glycoprotein synthesis, scale-up of these processes is challenging and an area of intense research activity (see Chapter 49).
GLYCAN THERAPEUTIC APPROACHES TO METABOLIC DISEASES
Salvage versus De Novo Synthesis
All monosaccharides needed for cellular glycan synthesis can be obtained from glucose through metabolic interconversions (see Chapter 4). Alternatively, monosaccharides can be derived from the diet or salvaged from degraded glycans. The relative contributions of different sources can vary with the cell type. For instance, even though all mammalian cells use sialic acid, only some contain high amounts of UDP-GlcNAc epimerase/N-acetylmannosamine kinase (GNE), which is required for the de novo synthesis of CMP-sialic acid. But sialic acid salvage from degraded glycans is quite efficient, decreasing the demand on the de novo pathway. Similarly, galactose, fucose, mannose, N-acetylglucosamine, and N-acetylgalactosamine can come from diet or be salvaged for glycan synthesis, whereas glucuronic acid, iduronic acid, and xylose cannot. All monosaccharides derived from diet or degraded glycans can be catabolized for energy, and, again, cells vary in their reliance on the different pathways.
The variable contributions of these pathways are important for therapy of some diseases. Patients with congenital disorder of glycosylation type Ib (CDG-Ib), who are deficient in phosphomannose isomerase, benefit greatly from oral mannose supplementation to bypass the insufficient supply of glucose-derived mannose 6-phosphate. A few CDG-IIc patients defective in fucose transporters have been treated with fucose to restore synthesis of sialyl-Lewis x on leukocytes (see Chapter 42). Some patients with Crohn's disease show clinical improvement with oral N-acetylglucosamine supplementation, but the mechanism is unknown. Mice deficient in GNE activity have kidney failure, but providing N-acetylmannosamine in the diet prevents this outcome. Clinical trials using N-acetylmannosamine to treat GNE-deficient patients with hereditary inclusion body myopathy type II (HIBM-II) have been conducted but have yielded inconclusive results.
Special Diets
Some monosaccharides and disaccharides can be toxic to humans who lack specific catabolizing enzymes. For example, people who lack fructoaldolase (aldolase B) accumulate fructose-1-phosphate, which ultimately causes ATP depletion and disrupts glycogen metabolism. Prolonged fructose exposure in these people can be fatal, and fructose-limited diets are critical. Deficiencies in the ability to metabolize galactose (see Chapter 4) are mostly due to a severe reduction in galactose-1-phosphate uridyl transferase activity and cause galactosemia. Although these patients are asymptomatic at birth, ingesting milk leads to vomiting and diarrhea, cataracts, hepatomegaly, and even neonatal death. Low-galactose or galactose-free diets can prevent these life-threatening symptoms. However, even these diets do not prevent unexplained long-term complications, which include speech and learning disabilities and ovarian failure in females with galactosemia.
Infants hydrolyze lactose (Galβ1-4Glc) quite well, but the level of intestinal lactase catalyzing the breakdown of lactose can be much lower or absent in adults because of down-regulation of lactase gene expression after childhood. About two-thirds of the human population has lactase nonpersistence, making milk products a dietary annoyance. Unabsorbed lactose provides an osmotic load and is metabolized by colonic bacteria, causing diarrhea, abdominal bloating, flatulence, and nausea. Lactase persistence has evolved in certain pastoral populations from northwestern Europe, India, and Africa, allowing milk consumption in adult life. However, many adults either avoid lactose-containing foods or use lactase tablets to improve lactose digestion.
Substrate Reduction Therapy
The failure to turn over glycans by lysosomal degradation driven by a plethora of glycoside hydrolases causes serious problems for patients with lysosomal storage disorders. Deficiencies in individual lysosomal enzymes or their trafficking lead to pathological accumulation of their substrates in inclusion bodies inside the cells (see Chapter 41). One approach to treating these disorders is to inhibit initial glycan synthesis, a strategy termed substrate reduction therapy (SRT). Reduced synthesis of the initial compound decreases the load on the impaired enzyme, and some patients show significant clinical improvement. A small-molecule drug used for SRT is N-butyldeoxynojirimycin (or N-butyl-DNJ) (miglustat, Zavesca), which was approved in 2002 to treat Gaucher's disease (glucocerebrosidase deficiency).
Lysosomal Enzyme Replacement Therapy
Another approach for treating lysosomal storage disorders is enzyme replacement therapy. Unlike most therapeutic glycoproteins that interact with target receptors on the surface of cells, lysosomal enzymes developed for replacement therapy must be delivered intracellularly to lysosomes, their site of action. During the normal biosynthesis of lysosomal enzymes, their N-glycans become modified with mannose 6-phosphate (Man-6-P) residues, which target them to lysosomes using Man-6-P receptors (see Chapter 30). The challenge for enzyme replacement therapy is to get the enzymes targeted properly to lysosomes, where they can degrade accumulated substrate. Enzyme replacement therapy for Gaucher's disease targets the lysosomes of macrophages via the cell-surface mannose receptor (see Chapter 31). The four recombinant enzyme products imiglucerase (approved in 1995), velaglucerase (approved in 2010), taliglucerase alfa (Elelyso, approved in 2012), and eliglustat (Cerdelga, approved in 2014) are marketed to treat Gaucher's disease.
The success of glucocerebrosidase treatment stimulated the development of lysosomal enzymes for treatment of other lysosomal storage diseases such as Fabry's disease, mucopolysaccharidoses type I, II, and VI, and Pompe's disease. The replacement therapies clearly have beneficial effects and prolong life but are extremely expensive.
Chaperone Therapy
A third approach for treating lysosomal storage disorders takes advantage of the fact that some genetic defects lead to misfolding of the encoded enzyme in the endoplasmic reticulum (ER). Low-molecular-weight competitive inhibitors of some of these enzymes can act as “chaperones,” which stabilize the folded enzyme in the ER and effectively rescue the mutation and increase the steady state concentration of active enzyme in the lysosome. The dose of the inhibitor must be carefully adjusted to ensure that the inhibitory effects on enzyme function do not overshadow beneficial effects on folding. Only a low level of enzyme restoration is needed to significantly reduce the accumulation of undigested glycan substrates, indicating that lysosomal hydrolases are normally present in large catalytic excess.
THERAPEUTIC APPLICATIONS OF GLYCOSAMINOGLYCANS
The use of purified glycans as therapeutics has received less attention than the development of glycoprotein-based treatments. Difficulties in establishing structure–activity relationships because of the large number of stereocenters and functional groups, undesirable pharmacokinetics of available formulations, poor oral absorption of the compounds, and low-affinity interactions with drug targets have limited their development. Some successful glycan drugs, such as the anticoagulant heparin, are given by injection, although efforts are under way to convert heparin into an orally absorbable form by complexing it with positively charged molecules. It may be possible to deliver other hydrophilic and/or negatively charged glycan drugs in this way to allow penetration of the intestinal barrier. Glycans are also sometimes attached to hydrophobic drugs to improve the drugs’ solubility and ability to cross biological membranes to enter cells and alter their pharmacokinetics.
The anticoagulant heparin is, as discussed in Chapters 16, 43, and 46, one of the most widely prescribed drugs today. Heparin binds and activates antithrombin, a protease inhibitor of the coagulation cascade. Antithrombin activation leads to rapid inhibition of thrombin and factor Xa, shutting down the production of fibrin clots. Billions of doses of heparin (several metric tons) are produced by autodigestion of pig intestines, followed by graded fractionation of the products. Unfractionated heparin produces a variable anticoagulant response as it also binds to several plasma, platelet, and endothelial proteins. Low-molecular-weight (LMW) heparins are derived from chemical or enzymatic cleavage of heparin to form smaller fragments. The pharmacological properties and the relative efficacy of the various LMW heparins are superior to those of unfractionated heparin and fewer secondary complications are reported. LMW heparins have replaced unfractionated heparins as therapeutic of choice in virtually all developed countries. In price-sensitive markets the unfractionated products are still heavily used. The preparation of recombinant heparin based on heparin biosynthesis enzymes is still under development. Arixtra, a synthetic heparin pentasaccharide that binds antithrombin exactly as isolated heparin is used to prevent deep-vein thrombosis and pulmonary embolism and has gained market share in recent years even though it is more costly.
To prevent excessive bleeding, rapid neutralization of heparin is desirable. Administration of the basic protein protamine, which binds to heparin, neutralizes its activity, and results in clearance of the complex by the kidney and liver. Heparin is also used to treat protein-losing enteropathy (PLE), likely working by competing for proinflammatory heparin-binding cytokines that trigger PLE in susceptible patients (see Chapter 43).
Hyaluronan (see Chapter 15) is a naturally occurring glycosaminoglycan that is extensively used in surgical applications. Because of its viscoelastic properties, hyaluronan has lubricating and cushioning properties that have made it useful for protecting the corneal endothelium during ocular surgery. Hyaluronan is also useful in postsurgical wound healing. The mechanism of action is not well-understood, but may involve hyaluronan-binding proteins that mediate cell adhesion (see Chapter 15). Intra-articular injections of hyaluronan are used to treat knee and hip osteoarthritis. Modest improvement in patients treated with hyaluronan, may be the result of a mechanical (as a viscosupplement) and/or a biological (via signaling pathways) effect. Hyaluronan is used in very large quantities as a tissue filler in cosmetic medicine.
GLYCONUTRIENTS
“Glyconutrient” is a term used by the nutritional supplement industry to describe some of their products with wide-ranging claims concerning potential benefits. In most cases, these claims have not been substantiated through placebo-controlled, double-blind trials with defined, quantifiable outcomes. Much work is needed in this area to obtain insight into the potential role of dietary glycans on human health and to help consumers make informed decisions regarding their use.
Mixtures of plant polysaccharides such as larch bark arabinogalactan and glucomannan are often termed “glyconutrients” that are claimed to contain “essential monosaccharides” needed for “cell communication.” Because all monosaccharides can be made in vivo from glucose (except in patients with rare genetic deficiencies; see Chapter 42), none of the other monosaccharides are actually known to be “essential.” Moreover, arabinogalactan and glucomannan are not degraded to available monosaccharides in the stomach or small intestine. Instead, anaerobic bacteria in the colon metabolize them and produce short-chain fatty acids. No peer-reviewed clinical studies support the efficacy of such “glyconutrients” for any disease or condition. Nevertheless, the following examples demonstrate how dietary glycans might have beneficial effects.
Glucosamine and Chondroitin Sulfate
Glucosamine (often mixed with chondroitin sulfate) has been promoted to relieve symptoms of osteoarthritis, which involves the age-dependent erosion of articular cartilage. Cartilage provides a cushion between the bones to minimize mechanical damage, and a net loss of cartilage occurs when the degradation rate exceeds the synthetic rate. Claims that glucosamine improves osteoarthritis symptoms and restores partially the structure of the eroded cushion in the knees are controversial. Superficially, this would seem to make sense, because primary glycans of cartilage include hyaluronan (see Chapter 15) and chondroitin sulfate, both of which contain hexosamines within their structure (see Chapter 16). Nevertheless, veterinarians report positive results after treating animals with glucosamine for more than two decades.
Positive effects of chondroitin sulfate on osteoarthritis are not well-documented. It remains unclear how the acidic chondroitin sulfate polymer can be absorbed and delivered to its proposed site of action.
Xylitol and Sorbitol in Chewing Gum
Some studies suggest that chewing gum containing sugar alditols, such as xylitol and sorbitol, can help control the development of dental caries. The benefit of these reduced sugars seems to be based on stimulation of salivary flow and an antimicrobial effect by inhibiting a glucosyltransferase that blocks glucose utilization by Streptococcus mutans. Xylitol also inhibits the expression and secretion of proinflammatory cytokines from macrophages and inhibits the growth of Porphyromonas gingivalis, one of the suspected causes of periodontal disease.
Milk Oligosaccharides
Human milk contains ∼70 g/L of lactose and 5–10 g/L of free oligosaccharides. More than 130 different glycan species have been identified with lactose at the reducing end, including poly-N-acetyllactosamine units. Some glycans are α2-3- and/or α2-6-sialylated and/or fucosylated in α1-2, α1-3, and/or α1-4 linkages. In contrast, bovine milk, the typical mainstay in human infant formulas, contains much smaller amounts of fucose oligosaccharides. These differences may account for some of the physiological advantages seen for breastfed versus formula-fed infants. The glycans may also favor growth of a nonpathogenic bifidogenic microflora and/or block pathogen adhesion that causes infections and diarrhea. Surprisingly, a substantial number of human milk oligosaccharides remain almost undigested in the infant's intestine and are excreted intact into the urine. Whether supplementing infant formula with specific, biologically active free glycans enhances infant health is unknown.
GLYCANS AS VACCINE COMPONENTS
Microbial Vaccines
Polysaccharide vaccines consisting solely of glycan components typically elicit poor immunity, especially in infants. Since glycans are T-cell-independent antigens they do not effectively stimulate T-helper-dependent activation and class switching of B-cell-mediated immunity. Conjugate vaccines consisting of glycans coupled to carrier proteins have proven to be highly effective. Three major conjugate vaccines are marketed today: Haemophilus influenzae type b (Hib) causes an acute lower respiratory infection among young children that constitute a high-risk group for H. influenzae type B infections. Consequently, the Hib polysaccharide vaccine introduced in 1985 was withdrawn from the market in 1988 and replaced by capsular polysaccharide (CPS-)protein conjugate vaccine formulations. A conjugated form of a Hib-derived oligosaccharide coupled to a protein carrier is part of routine vaccination schedules and has been so successful that infectious diseases caused by this bacterium are nearly eradicated in vaccinated populations. The first semisynthetic glycoconjugate vaccine QuimiHib marketed in Cuba contains glycan chains with an average length of 16 monosaccharide residues. Pneumococcal conjugate vaccines have been developed to cover an increasing number of serotypes, and current formulations are 10- (Synflorix, GlaxoSmithKline) and 13-valent (Prevnar13, Pfizer). Prevnar 13 provides protection against serotypes that account for >70% of cases of invasive pneumococcal disease worldwide and is the best-selling vaccine with revenues of six billion U.S. dollars in 2019. Conjugate vaccines to protect from Neisseria meningitides are also very successful on the market. Because of the fast onset and rapid progression of meningococcal infections, vaccination is required to protect against this disease. Several conjugated CPS vaccines are licensed in different parts of the world: The tetravalent serogroup A, C, W, and Y (Menactra, Menveo, and Nimenrix) and a few monovalent vaccines based on serogroup C CPS (Meningitec, Menjugate, NeisVac-C). Two combination vaccines for N. meningitidis and H. influenzae type b (Hib) are available against meningococcal serogroups C/Y (MenHibrix) and against meningococcal serogroup C (Menitorix). A monovalent serogroup A vaccine (MenAfriVac) is widely used in the sub-Saharan meningitis belt of Africa.
Currently, several new vaccines based on synthetic oligosaccharide antigens are being developed to protect children and the elderly from a variety of bacterial infections. Vaccines to protect from hospital acquired infections caused by Clostridium difficile and Klebsiella pneumoniae that are increasingly antibiotic resistant are in preclinical evaluation.
Cancer Vaccines
Several carbohydrate-based cancer vaccines are at different stages of development to treat cancer. Ganglioside immunogens present on certain types of cancer cells such as gangliosides GM2 and GD2 in melanomas and Globo H in breast cancer are being explored. Treatment strategies targeting the shorter glycan sequences such as sialyl-Tn (sialylα2-6GalNAcα-) found on cancer mucins (see Chapter 44) have seen little progress in 20 years. The synthetic Globo H hexasaccharide (see Figure 57.1) resembling the breast and prostate cancer antigen reached Phase 3 clinical trials but failed to gain marketing approval. Further human clinical trials are ongoing. Instead of active immunization of immunocompromised patients, the use of humanized anti-glycan antibodies (passive immunization) is now explored in preclinical evaluations.
BLOCKING GLYCAN RECOGNITION IN DISEASE
Blocking Infection
As discussed in Chapter 34, many microbes and toxins bind to mammalian tissues by recognizing specific glycan ligands. Thus, small soluble glycans or glycan mimetics can be used to block the initial attachment of microbes and toxins to cell surfaces (or block their release), and thus prevent or suppress infection. Because many of these organisms naturally gain access through the airways or gut, the glycan-based drugs can be delivered directly without being distributed systemically. Milk oligosaccharides are natural antagonists of intestinal infection in infants (see above). Glycosylated polymers block the binding of viruses such as influenza. Although backed by a strong scientific rationale and robust in vitro studies, such “antiadhesive” or “mimicry” therapies have not yet found much practical application.
Inhibition of Selectin-Mediated Leukocyte Trafficking
When specific glycan-protein interactions are responsible for selective cell–cell interactions and a resulting pathology, then administration of small-molecule glycomimetics of the natural ligand is a useful means of intervention. Selectin-mediated recruitment of neutrophils and other leukocytes into sites of inflammation or ischemia/reperfusion injury involves specific selectin–glycan interactions in the vascular system (see Chapters 31 and 46). The use of sialyl-Lewis x tetrasaccharide derivatives failed because of poor oral availability and a short serum half-life. Glycomimetics that preserve the essential functionality of the parent tetrasaccharide but eliminate unwanted polar functional groups and synthetically cumbersome glycan components have been successful. The design of a monosaccharide glycomimetic starting from sialyl-Lewis x is shown in Figure 57.3. First, the sialic acid residue was replaced with a charged glycolic acid group, the N-acetylglucosamine residue was then replaced with an ethylene glycol linker, and finally the galactose residue was replaced with a linker moiety. The resulting glycomimetic had E-selectin binding affinity comparable to sialyl-Lewis x.
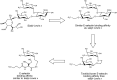
FIGURE 57.3.
Glycomimetic E-selectin inhibitors based on sialyl-Lewis x.
GMI-1070, targeting E-, P-, and L-selectin, yielded unconvincing results in Phase 3 clinical trials as a treatment for sickle cell crisis and is under further investigation.
TRANSFUSION AND TRANSPLANTATION REJECTION BY ANTIGLYCAN ANTIBODIES
A variety of glycans, including the classical A and B blood group determinants, can act as barriers to blood transfusion and transplantation of organs (Chapters 13 and 46). Rejection of mismatched blood or organs occurs because hosts have a high titer of preexisting antibodies against the glycan epitopes, presumably as a prior reaction to related structures found on bacteria or other microbes. In the case of the ABO blood groups, incompatibility is routinely managed by blood and tissue typing and finding an appropriate donor for the recipient. Bacterial enzymes can be used in vitro to remove the A and B blood group determinants from A and B red cells, converting them into “universal donor” O red cells.
A related problem is found in xenotransplantation (i.e., the transplantation of organs between species), which is actively being pursued as a solution for the shortage of human organs for patients. The animal donors of preference are pigs, because many porcine organs resemble those of humans in size, physiology, and anatomy. However, unlike humans and certain other primates, pigs and most other mammals produce the terminal “α-Gal” epitope on glycoproteins and glycolipids. Because humans have naturally occurring high-titer antibodies in blood directed toward this epitope, this results in hyperacute rejection of porcine organ transplants, via reaction of the antibodies with endothelial cells of blood vessels. Attempts to prevent this reaction, include blood filtration over glycan affinity columns to remove xenoreactive antibodies and blockade of the interaction by infusing soluble competing oligosaccharides. Transgenic pigs lacking the reactive epitope have also been produced, as have animals with an excess of complement-controlling proteins on their cell surfaces. Pig organs also have high levels of the nonhuman sialic acid (Neu5Gc), against which most humans have antibodies. Even if this problem is solved, there are other glycan and protein structural differences between humans and pigs that cause later stages of graft rejection, thus necessitating immunosuppression.
ACKNOWLEDGMENTS
The authors acknowledge helpful comments and suggestions from Morten Thaysen-Andersen.
FURTHER READING
- Kunz C, Rudloff S, Baier W, Klein N, Strobel S. 2000. Oligosaccharides in human milk: structural, functional, and metabolic aspects. Annu Rev Nutr 20: 699–722. doi:10.1146/annurev.nutr.20.1.699 [PubMed: 10940350] [CrossRef]
- Gomord V, Chamberlain P, Jefferis R, Faye L. 2005. Biopharmaceutical production in plants: problems, solutions and opportunities. Trends Biotechnol 23: 559–565. doi:10.1016/j.tibtech.2005.09.003 [PubMed: 16168504] [CrossRef]
- Joshi L, Lopez LC. 2005. Bioprospecting in plants for engineered proteins. Curr Opin Plant Biol 8: 223–226. doi:10.1016/j.pbi.2005.01.003 [PubMed: 15753005] [CrossRef]
- Pastores GM, Barnett NL. 2005. Current and emerging therapies for the lysosomal storage disorders. Expert Opin Emerg Drugs 10: 891–902. doi:10.1517/14728214.10.4.891 [PubMed: 16262569] [CrossRef]
- Beck M. 2007. New therapeutic options for lysosomal storage disorders: enzyme replacement, small molecules and gene therapy. Hum Genet 121: 1–22. doi:10.1007/s00439-006-0280-4 [PubMed: 17089160] [CrossRef]
- Brown JR, Crawford BE, Esko JD. 2007. Glycan antagonists and inhibitors: a fount for drug discovery. Crit Rev Biochem Mol Biol 42: 481–515. doi:10.1080/10409230701751611 [PubMed: 18066955] [CrossRef]
- Eklund EA, Bode L, Freeze HH. 2007. Diseases associated with carbohydrates/glycoconjugates. In Comprehensive glycoscience (ed. Kamerling JP, et al., editors. ), Vol. 4, pp. 339–372. Elsevier, New York. doi:10.1016/b978-044451967-2/00098-2 [CrossRef]
- Hamilton SR, Gerngross TU. 2007. Glycosylation engineering in yeast: the advent of fully humanized yeast. Curr Opin Biotechnol 18: 387–392. doi:10.1016/j.copbio.2007.09.001 [PubMed: 17951046] [CrossRef]
- Schultz BL, Laroy W, Callewaert N. 2007. Clinical laboratory testing in human medicine based on the detection of glycoconjugates. Curr Mol Med 7: 397–416. doi:10.2174/156652407780831629 [PubMed: 17584080] [CrossRef]
- von Itzstein M. 2007. The war against influenza: discovery and development of sialidase inhibitors. Nat Rev Drug Discov 6: 967–974. doi:10.1038/nrd2400 [PubMed: 18049471] [CrossRef]
- Schnaar RL, Freeze HH. 2008. A “glyconutrient sham”. Glycobiology 18: 652–657. doi:10.1093/glycob/cwm098 [PubMed: 17855741] [CrossRef]
- Ernst B, Magnani JL. 2009. From carbohydrate leads to glycomimetic drugs. Nat Rev Drug Discov 8: 661–677. doi:10.1038/nrd2852 [PMC free article: PMC7097102] [PubMed: 19629075] [CrossRef]
- Wilson RM, Dong S, Wang P, Danishefsky SJ. 2013. The winding pathway to erythropoietin along the chemistry–biology frontier: a success at last. Angew Chem Int Ed Engl 52: 7646–7665. doi:10.1002/anie.201301666 [PMC free article: PMC4729195] [PubMed: 23775885] [CrossRef]
- Bonam SR, Wang F, Muller S. 2019. Lysosomes as a therapeutic target. Nat Rev Drug Discov 18: 923–948. doi:10.1038/s41573-019-0036-1 [PMC free article: PMC7097195] [PubMed: 31477883] [CrossRef]
- Seeberger PH. 2021. Discovery of semi- and fully-synthetic carbohydrate vaccines against bacterial infections using a medicinal chemistry approach. Chem Rev 121: 3598–3626. doi:10.1021/acs.chemrev.0c01210 [PMC free article: PMC8154330] [PubMed: 33794090] [CrossRef]
- Smith BAH, Bertozzi CR. 2021.The clinical impact of glycobiology: targeting selectins, Siglecs and mammalian glycans. Nat Rev Drug Discov 20: 217–243; 244. doi:10.1038/s41573-020-00093-1 [PMC free article: PMC7812346] [PubMed: 33462432] [CrossRef]
- GLYCANS AS COMPONENTS OF SMALL-MOLECULE DRUGS
- THERAPEUTIC GLYCOPROTEINS
- GLYCOSYLATION ENGINEERING
- GLYCAN THERAPEUTIC APPROACHES TO METABOLIC DISEASES
- THERAPEUTIC APPLICATIONS OF GLYCOSAMINOGLYCANS
- GLYCONUTRIENTS
- GLYCANS AS VACCINE COMPONENTS
- BLOCKING GLYCAN RECOGNITION IN DISEASE
- TRANSFUSION AND TRANSPLANTATION REJECTION BY ANTIGLYCAN ANTIBODIES
- ACKNOWLEDGMENTS
- FURTHER READING
- Review Glycans in Biotechnology and the Pharmaceutical Industry.[Essentials of Glycobiology. 2015]Review Glycans in Biotechnology and the Pharmaceutical Industry.Seeberger PH, Cummings RD. Essentials of Glycobiology. 2015
- Review Glycans in Biotechnology and the Pharmaceutical Industry.[Essentials of Glycobiology. 2009]Review Glycans in Biotechnology and the Pharmaceutical Industry.Bertozzi CR, Freeze HH, Varki A, Esko JD. Essentials of Glycobiology. 2009
- Review Marine glycan-derived therapeutics in China.[Prog Mol Biol Transl Sci. 2019]Review Marine glycan-derived therapeutics in China.Gao Y, Zhang L, Jiao W. Prog Mol Biol Transl Sci. 2019; 163:113-134. Epub 2019 Mar 26.
- Review Structures Common to Different Glycans.[Essentials of Glycobiology. 2009]Review Structures Common to Different Glycans.Stanley P, Cummings RD. Essentials of Glycobiology. 2009
- Review Helminth Glycans at the Host-Parasite Interface and Their Potential for Developing Novel Therapeutics.[Front Mol Biosci. 2021]Review Helminth Glycans at the Host-Parasite Interface and Their Potential for Developing Novel Therapeutics.Bunte MJM, Schots A, Kammenga JE, Wilbers RHP. Front Mol Biosci. 2021; 8:807821. Epub 2022 Jan 10.
- Glycans in Biotechnology and the Pharmaceutical Industry - Essentials of Glycobi...Glycans in Biotechnology and the Pharmaceutical Industry - Essentials of Glycobiology
Your browsing activity is empty.
Activity recording is turned off.
See more...